Conceptual DFT Reactivity Descriptors Computational Study of Graphene and Derivatives Flakes: Doped Graphene, Graphane, Fluorographene, Graphene Oxide, Graphyne, and Graphdiyne
DOI:
https://doi.org/10.29356/jmcs.v64i3.1167Keywords:
Graphene, graphene derivatives, reactivity indexes, electronic structure calculations, conceptual DFTAbstract
Abstract. Allotropes of carbon such as graphene, graphane, fluorographene, doped graphene with N, B or P, graphene oxide, graphyne, and graphdiyne were studied through conceptual DFT reactivity descriptor indexes. To understand their chemical behavior and how they interact with different types of molecules, for instance, drugs (due to their potential use in drug carrier applications). This work shows the results of the changes in the global and local reactivity descriptor indexes and geometrical characteristics within the different graphene derivatives and rationalizes how they can interact with small molecules. Molecular hardness, the ionization energy, the electron affinity, electrodonating power index, and electroaccepting power indexes are the computed global reactivity descriptors. While, fukui functions, local softness, and molecular electrostatic potential are the local reactivity descriptors. The results suggest that the hybridization of carbons in the derivatives is kept close to sp3, while for graphene is sp2, the symmetry changes have as consequence changes in their chemical behavior. We found that doping with B or P (one or two atoms doped) and functionalizing with -OH or -COOH groups (as in graphene oxide), decreases the ionization energy in water solvent calculations, allowing for easier electron donation. On the other hand, doping with N atoms and functionalizing with F atoms increases the electron affinity. These types of changes enhance the chemisorption or physisorption by non-covalent interactions and covalent interactions with small molecules, principally, in the carbon atoms nearest to the doped/functionalized atom.
Resumen. Los alótropos de carbono como el grafeno, el grafano, el fluorografeno, el grafeno dopado con N, B o P, el óxido de grafeno, el grafino y el grafidiino se estudiaron mediante los índices de los descriptores de reactividad de la DFT conceptual. Ello, para comprender su comportamiento químico y cómo interactúan con diferentes tipos de moléculas, por ejemplo, fármacos (debido a su posible uso en aplicaciones como transportadores de fármacos). Este trabajo muestra los resultados de los cambios en los índices de los descriptores de reactividad global y local y las características geométricas de los diferentes derivados de grafeno y, predice cómo podrián interactuar con moléculas pequeñas. La dureza molecular, la energía de ionización, la afinidad electrónica, el índice de potencia electrodonadora y electroaceptora son los descriptores DFT de reactividad global calculados. Mientras que las funciones de fukui, la suavidad local y el potencial electrostático molecular son los descriptores de reactividad local. Los resultados sugieren que la hibridación de los carbonos en los derivados se mantiene cerca de sp3, mientras que para el grafeno es sp2, los cambios de simetría tienen como consecuencia cambios en su comportamiento químico. Descubrimos que el dopaje con B o P (uno o dos átomos dopados) y la funcionalización con grupos -OH o -COOH (como en el óxido de grafeno), disminuye la energía de ionización en los cálculos de solvente con agua, lo que permite una donación de electrones más fácil. Por otro lado, el dopaje con átomos de N y la funcionalización con átomos de F aumenta la afinidad electrónica. Estos tipos de cambios mejoran la quimisorción o fisisorción por interacciones no covalentes e interacciones covalentes con moléculas pequeñas, principalmente en los átomos de carbono más cercanos al átomo dopado/funcionalizado.
Downloads
References
Falcao, E. H. L.; Wudl, F. J. Chem. Technol. Biotechnol. 2007, 82, 524–531. https://doi.org/10.1002/jctb.1693
Yola, M. L. Curr. Anal. Chem. 2019, 15, 159–165. https://doi.org/10.2174/1573411014666180320111246
Gu, H.; Tang, H.; Xiong, P.; Zhou, Z. Nanomaterials 2019, 9, 130. https://doi.org/10.3390/nano9010130
Kang, J.; Wei, Z.; Li, J. ACS Appl. Mater. Interfaces 2019, 11, 2692–2706. https://doi.org/10.1021/acsami.8b03338
Yeo, J.; Jung, G. S.; Martín-Martínez, F. J.; Beem, J.; Qin, Z.; Buehler, M. J. Adv. Mater. 2019, 1805665, 1–24. https://doi.org/10.1002/adma.201805665
Novoselov, K. S.; Geim, A. K.; Morozov, S. V; Jiang, D.; Zhang, Y.; Dubonos, S. V; Grigorieva, I. V; Firsov, A. A. Science. 2004, 306, 666–669. https://doi.org/10.1126/science.1102896
Geim, A. K.; Novoselov, K. S. Nat. Mater. 2007, 6, 183–191. https://doi.org/10.1038/nmat1849
Baughman, R. H.; Eckhardt, H.; Kertesz, M. J. Chem. Phys. 1987, 87, 6687–6699. https://doi.org/10.1063/1.453405
Cranford, S. W.; Brommer, D. B.; Buehler, M. J. Nanoscale 2012, 4, 7797–7809. https://doi.org/10.1039/C2NR31644G
Peng, Q.; Dearden, A. K.; Crean, J.; Han, L.; Liu, S.; Wen, X.; De, S. Nanotechnol. Sci. Appl. 2014, 7, 1–29. https://doi.org/10.2147/NSA.S40324
Zhang, W.; Wu, L.; Li, Z.; Liu, Y. RSC Adv. 2015, 5, 49521–49533. https://doi.org/10.1039/C5RA05051K
Shin, D.-W.; Kim, T. S.; Yoo, J.-B. Mater. Res. Bull. 2016, 82, 71–75. https://doi.org/10.1016/j.materresbull.2016.02.009
Yadav, R.; Dixit, C. K. J. Sci. Adv. Mater. Devices 2017, 2, 141–149. https://doi.org/10.1016/j.jsamd.2017.05.007
Agnoli, S.; Favaro, M. J. Mater. Chem. A 2016, 4, 5002–5025. https://doi.org/10.1039/C5TA10599D.
Chronopoulos, D. D.; Bakandritsos, A.; Pykal, M.; Zbo?il, R.; Otyepka, M. Appl. Mater. today 2017, 9, 60–70. https://doi.org/10.1016/j.apmt.2017.05.004
Singh, D. P.; Herrera, C. E.; Singh, B.; Singh, S.; Singh, R. K.; Kumar, R. Mater. Sci. Eng. C 2018, 86, 173–197. https://doi.org/10.1016/j.msec.2018.01.004
Lee, J.-U.; Yoon, D.; Cheong, H. Nano Lett. 2012, 12, 4444–4448. https://doi.org/10.1021/nl301073q.
Luo, B.; Liu, S.; Zhi, L. Small 2012, 8, 630–646. https://doi.org/10.1002/smll.201101396
Geerlings, P.; De Proft, F.; Langenaeker, W. Chem. Rev. 2003, 103, 1793–1874. https://doi.org/10.1021/cr990029p
Cortés Arriagada, D. J. Mol. Model. 2013, 19, 919–930. https://doi.org/10.1007/s00894-012-1642-6
Saha, B.; Bhattacharyya, P. K. RSC Adv. 2016, 6, 79768–79780. https://doi.org/10.1039/C6RA15016K
SreeHarsha, N.; Maheshwari, R.; Al-Dhubiab, B.E.; Tekade, M.; Sharma, M.C.; Venugopala, K.N.; Tekade, R.K.; Alzahrani, A.M. Int. J. Nanomedicine. 2019,14, 7419–7429. doi:10.2147/IJN.S211224
Janak, J. F. Phys. Rev. B 1978, 18, 7165–7168. https://doi.org/10.1103/PhysRevB.18.7165
Casida, M. E. Phys. Rev. B 1999, 59, 4694–4698. https://doi.org/10.1103/PhysRevB.59.4694
Parr, R. G.; Pearson, R. G. J. Am. Chem. Soc. 1983, 105, 7512–7516. https://doi.org/10.1021/ja00364a005
Parr, R. G.; Yang, W. J. Am. Chem. Soc. 1984, 106, 4049–4050. https://doi.org/10.1021/ja00326a036
Gázquez, J. L.; Cedillo, A.; Vela, A. J. Phys. Chem. A 2007, 111, 1966–1970. https://doi.org/10.1021/jp065459f
Yang, W.; Mortier, W. J. J. Am. Chem. Soc. 1986, 108, 5708–5711. https://doi.org/10.1021/ja00279a008
Yang, W.; Parr, R. G. Proc. Natl. Acad. Sci. 1985, 82, 6723–6726. https://doi.org/10.1073/pnas.82.20.6723
Frisch, M. J.; Trucks, G. W.; Schlegel, H. B.; Scuseria, G. E.; Robb, M. A.; Cheeseman, J. R.; Scalmani, G.; Barone, V.; Mennucci, B.; Petersson, G. A.; et al. Gaussian 09. Revision C.01, Gaussian, Inc, Wallingford CT. Gaussian, Inc.: Wallingford CT 2010.
Zhao, Y.; Truhlar, D. G. J. Chem. Phys. 2006, 125, 194101. https://doi.org/10.1063/1.237099
Francl, M. M.; Pietro, W. J.; Hehre, W. J.; Binkley, J. S.; Gordon, M. S.; DeFrees, D. J.; Pople, J. A. J. Chem. Phys. 1982, 77, 3654–3665. https://doi.org/10.1063/1.444267
Hirshfeld, F. L. Theor. Chim. Acta 1977, 44, 129–138. https://doi.org/10.1007/BF00549096.
Miertuš, S.; Scrocco, E.; Tomasi, J. Chem. Phys. 1981, 55, 117–129. https://doi.org/10.1016/0301-0104(81)85090-2
Barnard, A. S.; Snook, I. K. J. Chem. Phys. 2008, 128, 94707. https://doi.org/10.1063/1.2841366
Silva, A. M.; Pires, M. S.; Freire, V. N.; Albuquerque, E. L.; Azevedo, D. L.; Caetano, E. W. S. J. Phys. Chem. C 2010, 114, 17472–17485. https://doi.org/10.1021/jp105728p
Kuc, A.; Heine, T.; Seifert, G. Phys. Rev. B 2010, 81, 85430. https://doi.org/10.1103/PhysRevB.81.085430
Deng, J.-P.; Chen, W.-H.; Chiu, S.-P.; Lin, C.-H.; Wang, B.-C. Molecules 2014, 19, 2361–2373. https://doi.org/10.3390/molecules19022361
Puigdollers, A. R.; Alonso, G.; Gamallo, P. Carbon N. Y. 2016, 96, 879–887. https://doi.org/10.1016/j.carbon.2015.10.043
Pearson, R. G. Acc. Chem. Res. 1993, 26, 250–255. https://doi.org/10.1021/ar00029a004.
Peyghan, A. A.; Rastegar, S. F.; Hadipour, N. L. Phys. Lett. A 2014, 378, 2184–2190. https://doi.org/10.1016/j.physleta.2014.05.016
Ketabi, N.; Tolhurst, T. M.; Leedahl, B.; Liu, H.; Li, Y.; Moewes, A. Carbon N. Y. 2017, 123, 1–6. https://doi.org/10.1016/j.carbon.2017.07.037
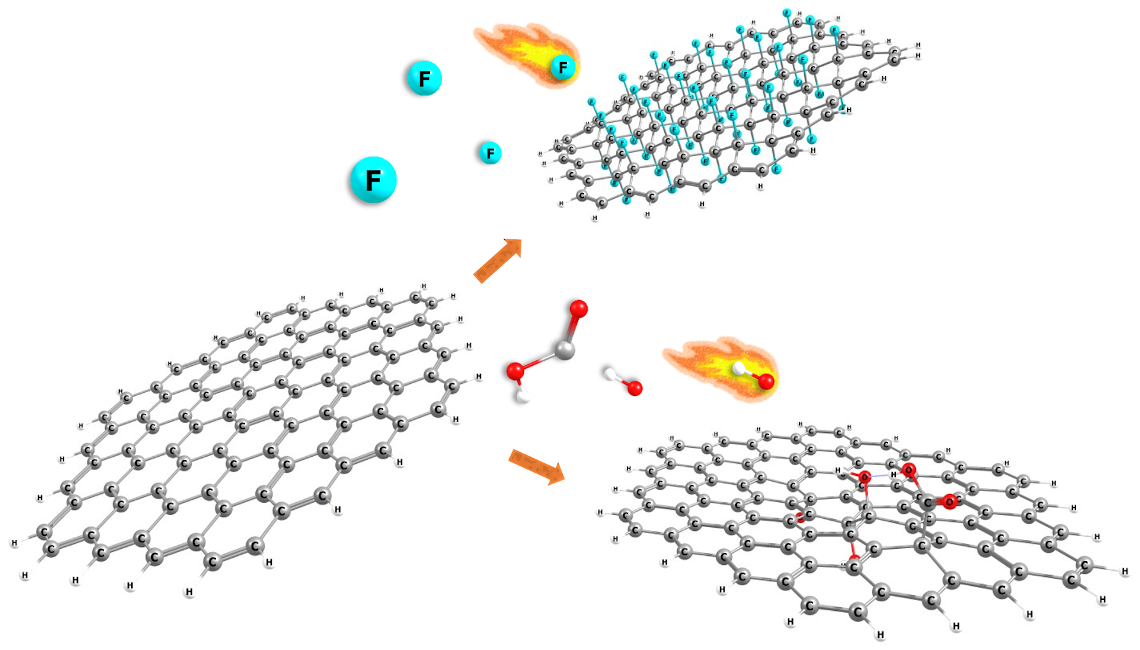
Downloads
Additional Files
Published
Issue
Section
License
Authors who publish with this journal agree to the following terms:
- Authors retain copyright and grant the journal right of first publication with the work simultaneously licensed under a Creative Commons Attribution License that allows others to share the work with an acknowledgement of the work's authorship and initial publication in this journal.
- Authors are able to enter into separate, additional contractual arrangements for the non-exclusive distribution of the journal's published version of the work (e.g., post it to an institutional repository or publish it in a book), with an acknowledgement of its initial publication in this journal.
